A wave is a type of movement (oscillation or periodic) that can transport energy from one point in space to another. You see waves in everyday life: Shaking the end of a rope transfers kinetic energy from your hand to the other end of the rope. When dropping a pebble into a pond causes waves to ripple outward across the water surface. And when lightning strikes, its makes sound waves (thunder) that travel through the expansion of air for miles. In all cases, kinetic energy travels through matter (the rope, water, or air) while the matter itself stays (mostly) in place.
An interesting example of waves occurs in sports stadiums when fans rise and stand with arms raised briefly before sitting down. Fans in neighboring sections likewise stand up and sit down in sequence. Despite the wave’s speed, none of the fans travel with the wave-they all stay in or above their seats.
Waves do not only travel through physical matter, some travel through air. Scientist James Clerk Maxwell discorved electromagtic waves, which included light. These waves have an oscillating electric field and a perpendicular magnetic field, both perpendicular to the direction of travel. These waves can travel through a vacuum at the speed of light (c) which is around a constant speed of 2.998×108 m/s.
Wave Properties
All waves, including forms of electromagnetic radiation, have a wavelength (λ), a frequency (ν) and an amplitude. We denote wavelength by λ, the lowercase Greek letter lambda, and frequency by ν, the lowercase Greek letter nu. The wavelength, shown below, is the distance between two consecutive peaks or troughs in a wave (measured in meters). Electromagnetic waves have wavelengths that fall within an enormous range-wavelengths of kilometers (103 m) to picometers (10−12 m).
Frequency measures how many wave cycles pass a point in a givent time, with one cycle being one complete waveleng (in the SI system, measured in seconds). The unit for frequency, expressed as cycles per second [s−1], is the hertz (Hz). Common multiples are megahertz (1 MHz = 1 × 106 Hz) and gigahertz (1 GHz = 1 × 109 Hz).
Amplitude is the wave’s height, which is half the distance beween peaks and troughs (shown below). Amplitude affects the wave intensity: for light is the brightness, and for sound is the loudness.
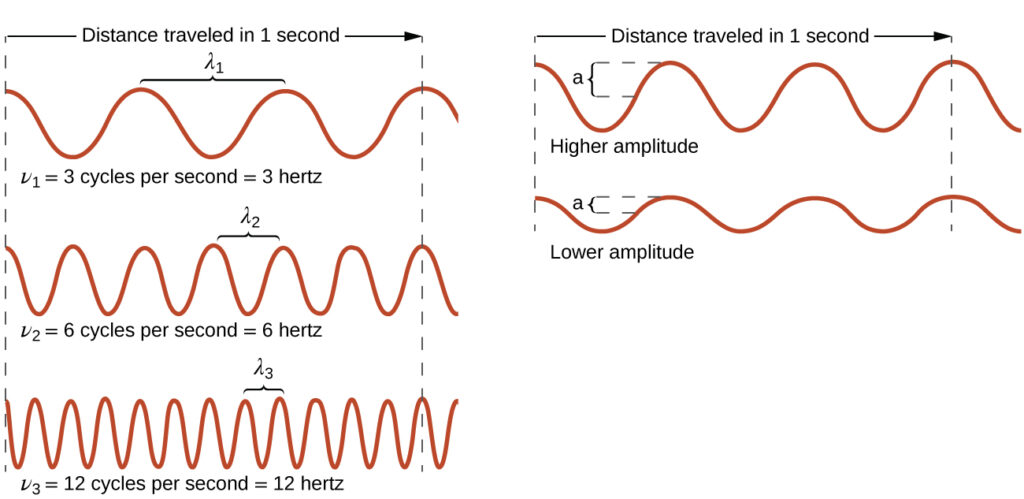
The product of a wave’s wavelength (λ) and its frequency (ν), λν, is the speed of the wave. Thus, for electromagnetic radiation in a vacuum, speed is equal to the fundamental constant, c:
$$c=2.998×10^8\; ms^{−1}=λν$$
Relationship Between Waves and Frequencies
Wavelength and frequency are inversely related: As the wavelength increases, the frequency decreases. The figure below shows this relationship and also the electromagnetic spectrum, the range of all types of electromagnetic radiation. Each color of visible light has specific frequencies and wavelengths associated with them. You can see that visible light makes up only a small portion of the electromagnetic spectrum, with each color having specific wavelength and frequency. The technologies developed for different parts of the electromagnetic spectrum require different units of measurement, for convinience and historical legacies. For example, radio waves use frequencies (MHz), while the visible region is usually specified in wavelengths (nm or angstroms).
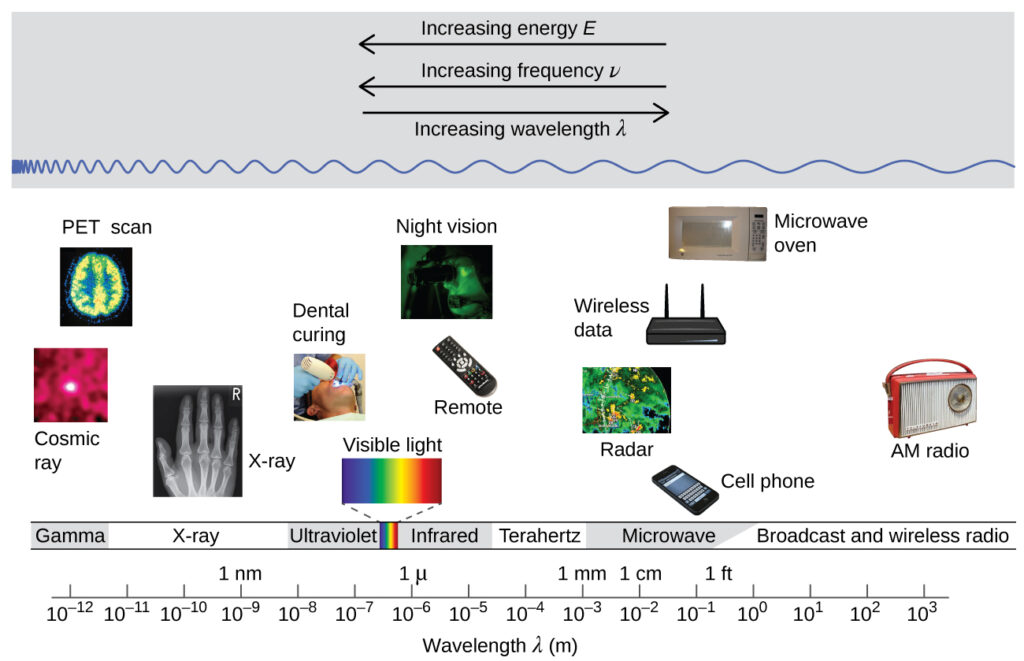
Determining Frequency and Wavelength
Determining the Frequency and Wavelength of Radiation
A sodium streetlight gives off yellow light that has a wavelength of 589 nm (1 nm = 1 × 10−9 m). What is the frequency of this light?
Solution
We can rearrange the equation c = λν to solve for the frequency:
$$ν=\frac{c}{λ}$$
Since c is expressed in meters per second, 589 nm are converted to meters.
$$ν=(\frac{2.998×10^8\; \require{enclose}\enclose{horizontalstrike}{m}s^{−1}}{589\; \enclose{horizontalstrike}{nm}})(\frac{1×10^9\; \enclose{horizontalstrike}{nm}}{1\; \enclose{horizontalstrike}{m}}) \\ \nu=5.09×10^{14}\; s^{−1}$$
Check Your Learning
One of the frequencies used to transmit and receive cellular telephone signals in the United States is 850 MHz. What is the wavelength in meters of these radio waves?
Answer:
0.353 m = 35.3 cm Wireless Communication
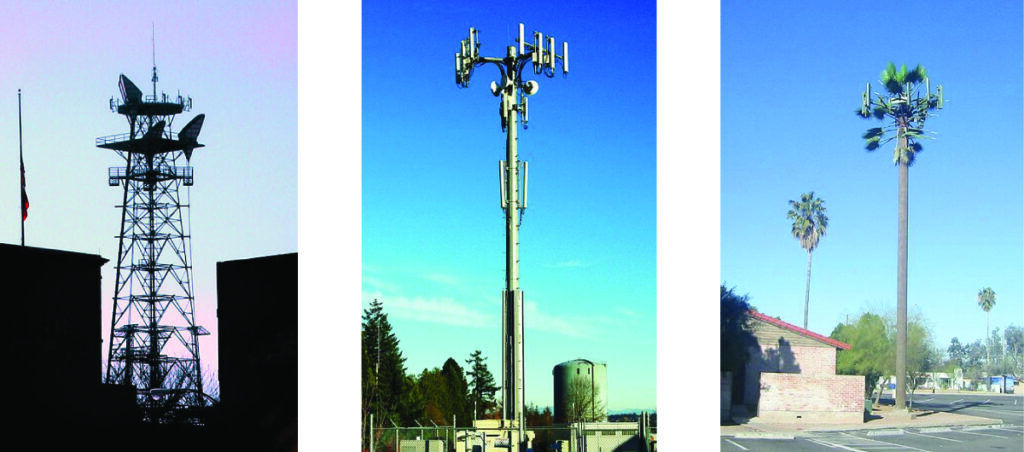
Radio Frequency Technolgies and Modulation Methods
Many valuable technologies ooperate in the radio (3 kHz-300 GHz) frequency region of the electromagnetic spectrum. At the low frequency (low energy, long wavelength) end of this region are AM (amplitude modulation) radio signals (540-2830 kHz) that can travel long distances. FM (frequency modulation) radio signals use higher frequencies (87.5-108.0 MHz). In AM radio, the transmission of information varies the amplitude of the wave (Figure 4 below). In FM radio, by contrast, the amplitude is constant and the instantaneous frequency varies.
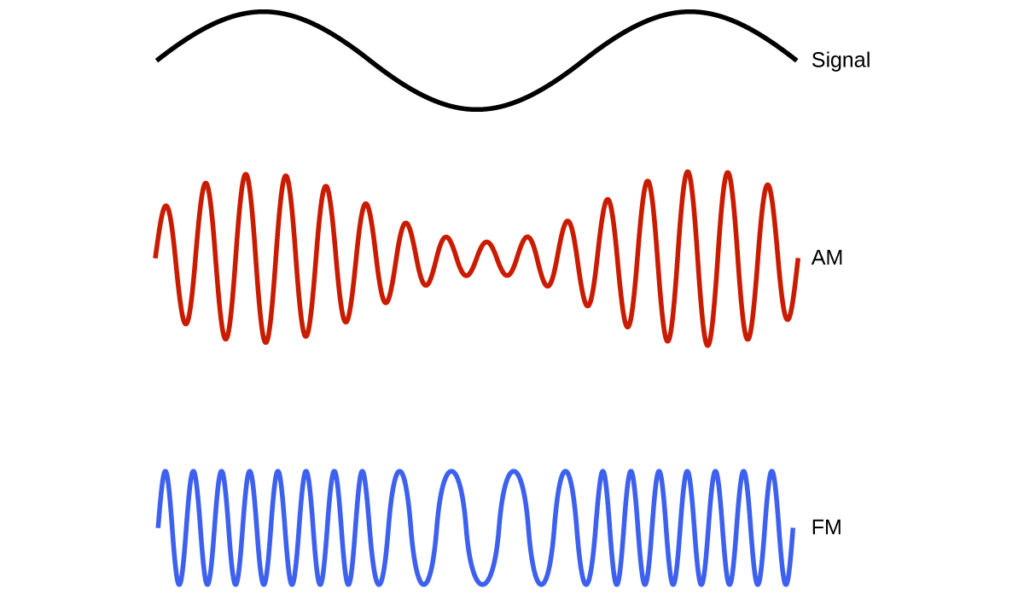
Other technologies also operate in the radio-wave portion of the electromagnetic spectrum. For example, 4G cellular telephone signals are approximately 880 MHz, while Global Positioning System (GPS) signals operate at 1.228 and 1.575 GHz, local area wireless technology (Wi-Fi) networks operate at 2.4 to 5 GHz, and highway toll sensors operate at 5.8 GHz. The applications use frequencies that are convenient because common building materials do not absorb such waves much.
Interference Patterns
One particularly characteristic phenomenon of waves results when two or more waves come into contact: They interfere with each other. Figure 5 shows the interference patterns that arise when light passes through narrow slits closely spaced about a wavelength apart. The wavelength affects fringe patterns, with shorter wavelength light producing more closely spaced fringes through a give set of slits. When the light passes through the two slits, each slit effectively acts as a new source, resulting in two closely spaced waves coming into contact at the detector (the camera in this case). The dark regions in Figure 5 correspond to regions where the peaks for the wave from one slit happen to coincide with the troughs for the wave from the other slit (destructive interference), while the brightest regions correspond to the regions where the peaks for the two waves (or their two troughs) happen to coincide (constructive interference).
Similarly, when two stones tossed close together into a pond, you can see interference patterns in the interactions between the waves produced by the stones. According to the laws of classical mechanics, particles moving cannot explain these patterns.

Portrait of a Chemist: Dorothy Hodgkin
Because the wavelengths of X-rays (10-10,000 picometers [pm]) are comparable to the size of atoms, X-rays can be used to determine the structure of molecules. When a beam of X-rays is passed through molecules packed together in a crystal, the X-rays collide with the electrons and scatter. Constructive and destructive interference of these scattered X-rays creates a specific diffraction pattern. Calculating backward from this pattern, the positions of each of the atoms in the molecule can be determined very precisely. One of the pioneers who helped create this technology was Dorothy Crowfoot Hodgkin.
She was born in Cairo, Egypt, in 1910, where her British parents were studying archeology. Even as a young girl, she was fascinated with minerals and crystals. When she was a student at Oxford University, she began researching how X-ray crystallography could be used to determine the structure of biomolecules. She invented new techniques that allowed her and her students to determine the structures of vitamin B12, penicillin, and many other important molecules. Diabetes, a disease that affects 382 million people worldwide, involves the hormone insulin. Hodgkin began studying the structure of insulin in 1934, but it required several decades of advances in the field before she finally reported the structure in 1969. Understanding the structure has led to better understanding of the disease and treatment options.